A groundbreaking career in plutonium science for national security
December 1, 2016
Albert MiglioriSeptember 1973. It’s late afternoon, the sky is black, and Jemez mountain lightning and thunder accompany a monsoon rain as I wind my way up the main hill road to Los Alamos for the first time, stunned by the view that confirms my decision to accept a Los Alamos Director’s Postdoctoral Fellow position. Of course, I am also terrified to be starting at a serious research institution. A few months earlier, I had completed my Ph.D. in condensed matter physics, and, as fate would have it, the Vietnam war had ended, releasing me from a two-year obligation to serve as a 1st Lieutenant in the U.S. Army Signal Corps on combat duty. I’d also just turned down a permanent position with another national laboratory, hoping to land the job at Los Alamos.
Graduate school had given me a superb education in hard-core, hands-dirty experimental physics, and I was hooked, then and now. During that first decade at Los Alamos, I had a blast. I was promoted to permanent staff and began working with John Wheatley, a famous low-temperature physicist, on acoustic engines—an idea I had from the operation of tuned exhausts in two-stroke motorcycle engines. Wheatley ran with this, and I was barely able to hang on, but this was how I got my start with acoustic methods.
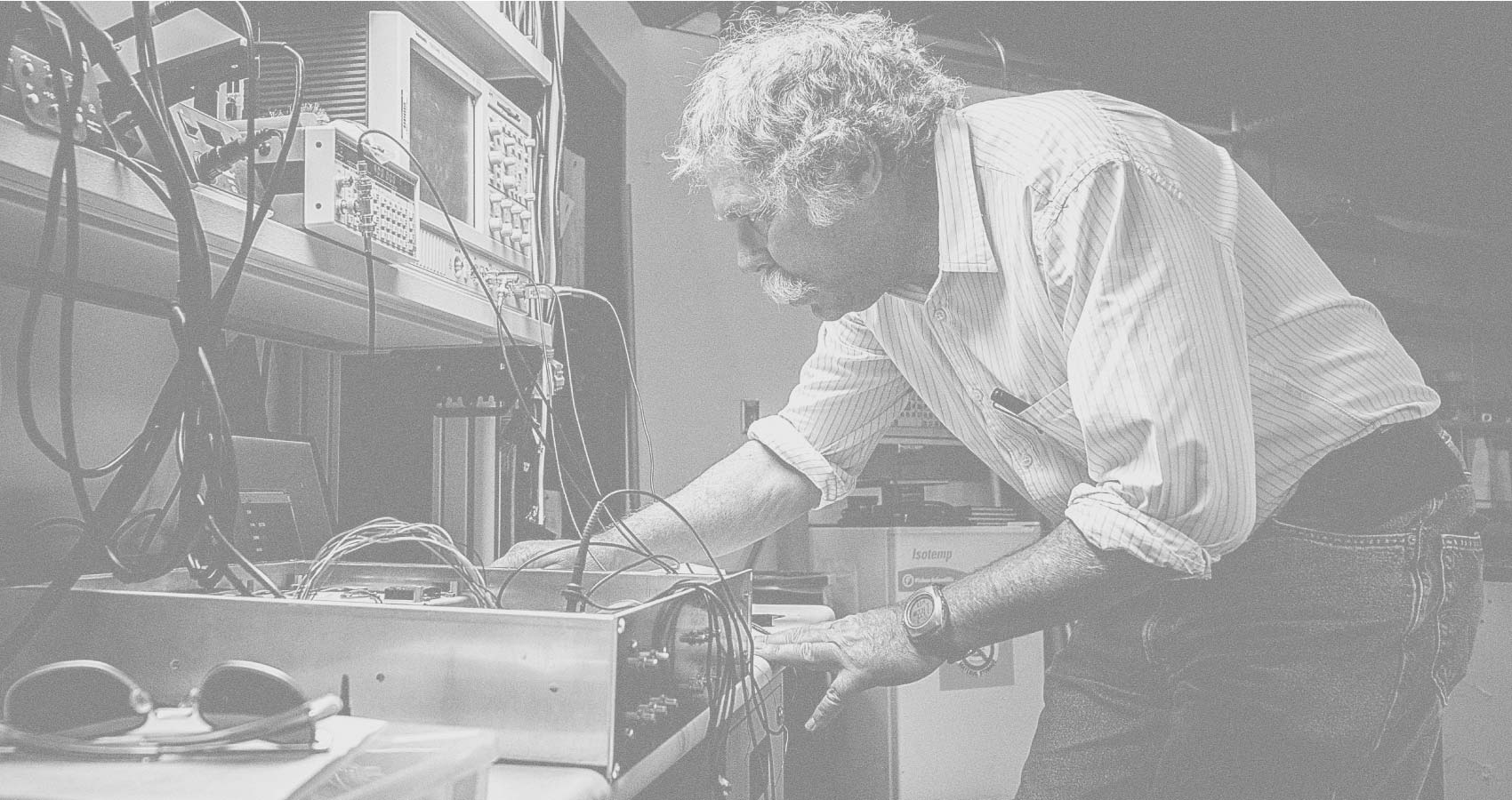
After Wheatley’s death, I headed back to condensed-matter physics. Around that same time, the physics world was turned upside down by the discovery of high-temperature superconductors. Unlike all previously discovered superconductors in which electrical current flows resistance-free below a critical temperature Tc close to absolute zero, high-Tc superconductors function at more accessible temperatures, perhaps someday without any cooling at all. (This would be nothing short of a technological revolution, and the quest for it continues to this day.)
With my doctoral work on superconductivity and the thermodynamics I had picked up since, I knew that a crucial high-Tc measurement would be of the superconductor’s bulk-modulus discontinuity at the superconducting transition—a required sharp change in the material’s elastic properties at Tc. So I began to develop a technique for carrying out that measurement, resonant ultrasound spectroscopy (RUS), not knowing at the time that geologists had been doing the same. Lucky for me (not so much for them), my background in electronics and Los Alamos’s advanced computing capabilities were such that my colleagues and I were able to develop this tool, hardware and software, to the point where it is now widely used. (In crediting the pioneering work by geologists that I only later found out about, I was quoted in Physics Today as saying, “Six months in the lab can save you a day in the library.”)
I am addicted to the visceral reaction of discovering something that, until that moment, no one on the entire planet knew.
Although a decade passed before we could get good enough high-Tc material to make the measurement, RUS became a useful ultrasound technique, and I was asked if I’d like to use it to measure plutonium. Talk about low-hanging fruit! The state of elastic stiffness measurements on plutonium was both a total mess and critically important to the Laboratory’s national security mission. And who could compete with us? So off I went to Tech Area 55 to see if plutonium rang like the bell we needed, which is what RUS actually measures—the ring frequencies of a solid object.
To our amazement, plutonium was an ideal RUS target; the first scan gave us all goosebumps, it was so good. During the next several years, we knocked off the elastic moduli over the full range of existence of several of plutonium’s crystal configurations: alpha, gamma, then beta. At the same time, we studied gallium-stabilized delta plutonium and, for all the measurements, got the error bars down to the size of the data points themselves. (Delta-phase plutonium, naturally occurring only well above room temperature, is useful because it is ductile, rather than brittle like the room-temperature alpha phase. A little added gallium brings the delta phase down to room temperature.)
Every few years along the way, I redesigned the electronics, getting better and better performance, until we realized that we had reached a noise-limited measurement precision of about one part in 108. We got there partly as a result of improved and home-built electronics and partly as a result of my colleague Boris Maiorov’s very clever way of backing out the effects of miniscule temperature variations so they didn’t mask our results. We could now see the key isotope of plutonium-239 aging, caused by accumulated damage from its own radioactivity, in real time over the course of hours! This meant that the difficulties in attempting to draw conclusions from accelerated aging mixes (with plutonium-238 added) could be worked around. It also meant that we could see the tiniest changes in phase of plutonium-gallium alloys and that we could obtain detailed, quantitative measurements of the thermodynamics, and hence connect our measurements to the material’s internal electronic structure and the equation of state that governs its macroscopic behavior.
With these successes, we had become the owners of two condensed-matter physics problems that perfectly fit the Los Alamos mission.
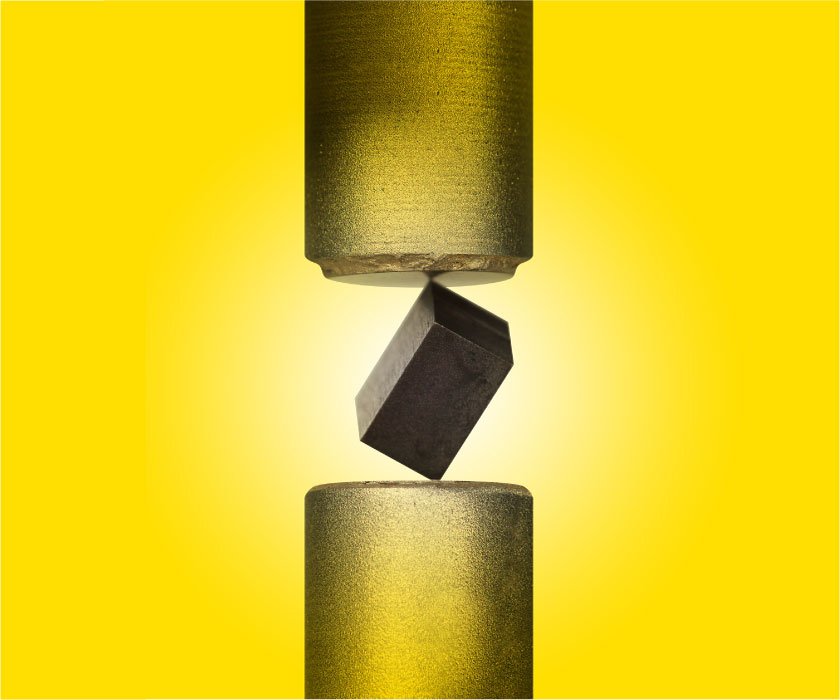
Problem 1: How do plutonium alloys age? We have a deep obligation to understand this; if we don’t, how can we be confident in the effectiveness of our weapons? We can’t just look at plutonium or look at a nuclear weapon. And we can’t test a weapon to see if it still works. Instead, we have to use all relevant science to understand everything that affects nuclear performance. (The fact that plutonium science turns out to be so enthralling in its own right is a tremendous bonus.)
Radiogenic byproducts of plutonium (helium, uranium, and more), radiation damage, and the thermodynamic stability of plutonium-gallium alloys all contribute to the aging process. Each has a knob we can adjust, such as temperature, gallium concentration, and even decay rate by incorporating longer-lived plutonium isotopes. We are now in the middle of this investigation, but already we know some things. Consider, for example, that each radioactive decay of a plutonium-239 atom delivers enough energy to raise about a million plutonium atoms above the melting point. And also consider that the decay constant (the thing that goes in exponentials when one calculates radioactive decays, a little longer than the half-life) for plutonium-239 is about a trillion seconds. Combining these, we expect that in about two weeks, every plutonium atom has been above the melting point, resetting a lot of age-related damage.
So one time scale for aging might be on the order of two weeks, which should be observable if the plutonium is new or the temperature has changed. When we change the temperature to look for this effect, we do indeed see that it takes about two weeks for the system to stabilize to a new, constant, and higher rate of change. Another time scale that we might conjecture would be much longer, based on the decay of plutonium-239 at a rate of 0.003 percent per year. At that rate, in about 350 years, 1 percent of the plutonium-239 is gone, and we can certainly expect to see a 1 percent change in properties. So 350 years might be another time scale (if we somewhat arbitrarily assign significance to the 1-percent level). That one we can’t address directly because we can’t wait that long, but, surprisingly, what we do see is that the rate of change of the bulk modulus of plutonium after being held at room temperature for about eight years is 0.2 percent per year—about 70 times faster than the decay rate. This rapid rate of change seems to me and my team likely to be caused by the accumulation of material defects from the melting and imperfect re-solidification following each atom’s decay.
Problem 2: What is the electronic structure of plutonium, meaning the actual arrangement and behavior of all its electrons? This has been a grand challenge in actinide science for decades. (Plutonium resides in the actinide series of the periodic table.) Constructing such an electronic-structure model would provide deep scientific insight, but would also provide tools to push the equation of state beyond where it can be measured, as well as better models for the thermal conductivity and heat capacity of hot plutonium and maybe even the liquid state—nice to know when making a plutonium casting.
We are now sitting on two breakthrough theories, one of which could finally explain plutonium’s electronic structure.
More than two decades ago, my colleague Per Soderlind at Lawrence Livermore National Laboratory suggested that if some sort of magnetic interactions were present, that could explain many of the mysteries of plutonium’s electronic structure. But magnetism had never been observed. In fact, a joint paper between the Institute for Transuranium Elements and Los Alamos insisted that there was no magnetism, and that had a chilling effect on what would turn out to be the correct theoretical approaches. However, in 2015, Los Alamos’s Marc Janoschek did indeed observe dynamic magnetism using neutron scattering, and a theory was constructed by his co-authors to explain it—including one of the authors of the now infamous paper claiming no magnetism [see “A Community of Electrons” in the October 2015 issue of 1663]. However, that theory was based solely on the neutron scattering results and therefore did not address other known properties.
To understand the difficulty plutonium presented to electronic structure theorists, and why this left a big gap, let’s examine the thermodynamics of a rubber band. When it is cold, a rubber band has lots of long-chain organic molecules with their bonds lined up in a straight line. When heated, the bond directions vibrate, so that they are no longer lined up. That produces bends in the long chains, making them shorter and thereby producing negative thermal expansion. Unlike a metal, you heat rubber and it gets smaller. That’s a general result, in the sense that to fight against the more probable outcome of an expanded solid (entropy benefit), there must exist a thermally accessible state that has a smaller volume when warm. This works for Invar, a zero-expansion iron alloy, and for more exotic materials like zirconium tungstate, where vibrating oxygen octahedra act just like the molecules in rubber.
However, our work on delta plutonium alloys with zero thermal expansion showed elastic softening upon warming that was an order of magnitude greater than that for, say, aluminum, which has a similar melting point. The trouble is, usually the higher-energy, smaller-volume state that blocks thermal expansion is stiffer, not softer (think of something being compressed). But Per and I realized right away that we could use his previously discredited model (the “Soderlind model”), the known thermal expansion (or lack thereof), and the energy value of Janoschek’s dynamic magnetism to compute the neutron scattering results, volume, and stiffness versus temperature.
We had no idea what the calculation would produce. If it made warmer delta plutonium stiffer, then it would be wrong. So we closed our eyes, hit enter on the computer, and waited for the massive computation to proceed. Well, something like that. And what we saw made us very happy. The calculation predicted a softening of delta plutonium with absolutely zero volume change, which is indeed what actually happens.
And we are now sitting on a breakthrough in the understanding of the fundamental electronic structure of plutonium. We have two theories in less than a year, constructed once the awful bottleneck of no plutonium magnetism was removed, with both Los Alamos and Lawrence Livermore involved. One theory gets several effects qualitatively correct, the other only applies to one measurement, but both are plausible. And those theories predict several other measurables, including the all-important Fermi surface—the state of the highest-energy electrons in the material, which can only be measured at the Los Alamos National High Magnetic Field Laboratory—as well as some magnetic and thermodynamic properties. Plutonium hasn’t been this exciting in a long time, with the promise of clear, hypothesis-driven measurements directed at validation of two new theories, one of which could finally explain plutonium’s electronic structure.
So, unlike my first few days at Los Alamos 43 years ago, I can see now many fascinating questions in physics, with answers essential to the Laboratory mission and to national security and with progress only possible in the team environment of a national lab. I am immensely privileged to be able to ask world-class scientists about things that confuse me and receive patient responses. I look back at successes in measurement science, plutonium, materials science, chemistry, and more. And I realize that I am addicted to the visceral reaction, rare and precious, of discovering something that until that moment, no one on the entire planet knew.
I’ll bet there’s a lot more to come.