Microscopes capture the three-dimensional movements of particles inside living cells
March 1, 2018
Rebecca McDonaldFollowing the inventions of microscopes and x-ray machines, constant technological advances have propelled humans’ ability to understand what is going on inside living organisms. But aside from an invasive endoscopic procedure, most methods of visualizing organs or cells produce only one- or two-dimensional still images, leaving much to be desired. For instance, to look at proteins and other cellular components, one must break open a cell and affix the proteins to a microscope slide or suspend them in a gel. To actually see how a protein moves around and functions with other cellular structures, scientists would instead need some kind of molecular video camera.
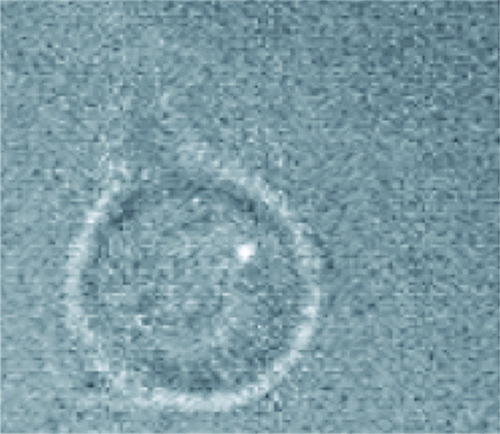
Over the last several years, many teams have been trying to invent one: developing new microscopes in an effort to capture the three-dimensional movements of particles inside live cells. In 2008, a Los Alamos team led by biophysicist Jim Werner developed an award-winning 3D-tracking microscope, one of only a few of its kind. Today, the scientists are still improving the technology, adding layers of data capture to help them truly understand molecular machinery at work. And with each advancement, they are getting closer to capturing the motion of life at a cellular level. Understanding such interactions and movements has a variety of potential applications, including medical ones, such as testing therapeutics.
The Los Alamos 3D-tracking microscope is based on the design of a typical confocal microscope, which uses a pinhole combined with an objective lens on either side of the specimen in an effort to increase resolution and eliminate out-of-focus light. By making the specimen glow and adding multiple detectors, the scientists can determine the 3D movement. The result is akin to the difference between just looking at an assembled car engine and actually watching the engine run. Instead of simply using microscopy to identify the presence and shape of cellular components, scientists are able to glean valuable spatial information about molecular interactions and overall function.
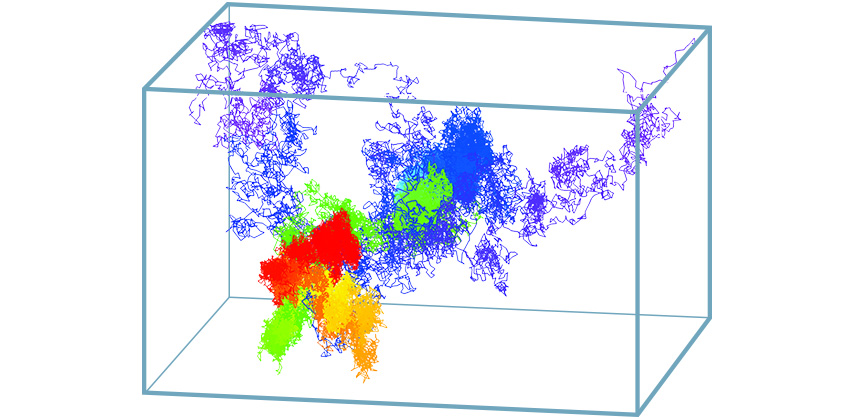
Werner explains that the molecule being tracked is fluorescently labeled (with a green fluorescent protein-type tag or a quantum dot) and positioned in the center of the probe volume so that the light it emits can be captured equally by four photodiode detectors. The detectors are arranged in a tetrahedron shape around the center of the sample in order to analyze the motion along multiple axes. As the labeled molecule moves away from the center, its distance from each detector becomes unequal and, using an algorithm, the team can estimate the new location of the molecule. Next, using the estimate of its current location, the scientists try to move the apparatus to re-center the molecule in the optical probe volume, and repeat the process approximately 200 times per second until they lose the object being tracked.
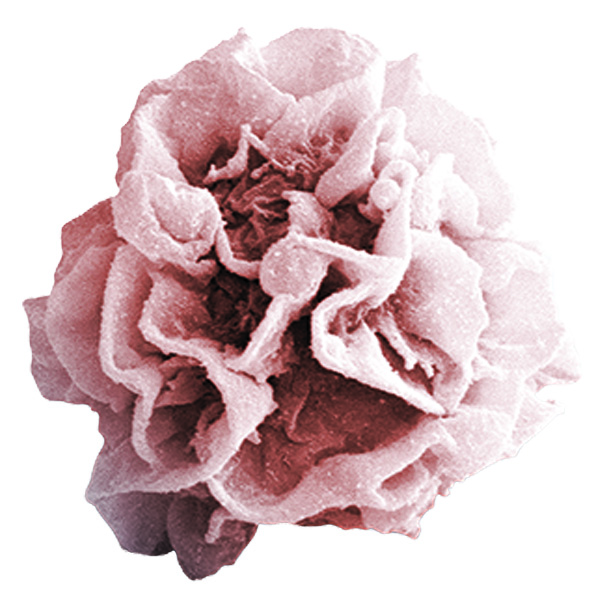
Using this microscope, Werner and his colleagues can track nanometer-sized (nm) particles at micrometer-per-second velocities (billionth and millionth of a meter, respectively) with a spatial accuracy that depends upon integration time (approximately 100 nanometers accuracy for a 3D position calculated from 5 milliseconds of data) for each of the x, y, and z axes. Along with various collaborators, the team has been able to observe many biological phenomena that could not otherwise be captured. For instance, by tracking the movements of quantum dots in a live cell, the team observed immune-system receptors in action as they initiated a cascade of signaling molecules. In a separate study, the scientists were able to depict the ruffled surface of a mast cell, a key player in allergies and anaphylaxis.
In recent experiments, the team added a specialized spinning disk with thousands of pinholes to the microscope to enable the capture of a still image of the whole cellular environment. This additional image provides an accurate location for where in the cell the tracked molecule’s motion is taking place, such as the approach of a protein to a receptor on a membrane. More recently, the team is expanding 3D tracking to new areas, including using the system for time-resolved super-resolution imaging and for studying other important 3D-transport problems outside of the biological sphere, such as how sand-like materials called proppants flow in 3D fracture networks important in hydraulic fracking. But for Werner, biological science and medical applications provide plenty of important targets for study.
“Understanding how, when, and why individual proteins interact in live cells is critical to understanding and predicting cell response, including when or why a cell becomes diseased, develops cancer, or dies due to exposure to infectious disease,” says Werner. “Furthermore, the insight about how proteins interact with cell membranes could potentially help us test the efficacy of getting therapeutic drugs into cells.” With innovative microscopy such as his 3D-tracking microscope, this understanding might be more attainable than ever before.